Most diamonds share a common origin story
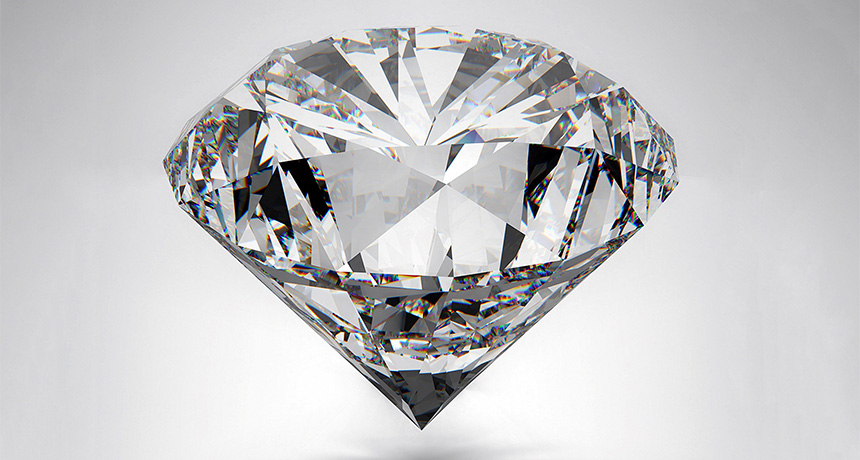
Even top-caliber diamonds aren’t perfect. And their imperfections are finally settling a debate about the origins of the gem-quality diamonds used in jewelry.
Previously, scientists had an explanation only for how cloudy and impurity-ridden fibrous diamonds form. Those diamonds crystallize inside fluid pockets deep within the Earth that contain compounds called carbonates. Carbonate-containing impurities inside fibrous diamonds provide information about the diamonds’ origins. Gem diamonds typically don’t contain these impurities, so scientists argued over whether the gems formed under different conditions than fibrous diamonds.
After an exhaustive hunt, geochemists have at last found microscopic impurities within gem-quality diamonds. These flaws suggest that pretty and ugly diamonds form from the same kinds of carbonate-containing fluids, the researchers report in the June 1 issue of Earth and Planetary Science Letters. The finding may also offer insights into the history of plate tectonics.
The work “gives us the first strong constraint on how gem diamonds grow,” says Thomas Stachel, a petrologist at the University of Alberta in Canada who was not involved in the research. “People had proposed various explanations for how these diamonds form, but it seems diamond formation is less diverse than we thought.”
Diamonds are made up of carbon atoms. At the pressures and temperatures found in the deep Earth, these carbon atoms can form a crystal structure. Rising magma then carries the crystals to the surface.
The type of diamonds prized for jewelry formed as early as 3.5 billion years ago. Fibrous diamonds date back only a few million years and formed more quickly. That quick creation trapped bits of surrounding material inside the crystal structure. Those inclusions suggest that these diamonds formed from the carbon atoms in carbonate-containing fluids. Gem-quality diamonds formed more slowly and usually don’t contain any inclusions. “That’s why they’re gem quality —there’s nothing in them,” says study coauthor Brooke Matat Jablon, a geochemist at the Hebrew University of Jerusalem.
Jablon and geochemist Oded Navon, also at the Hebrew University, hunted for inclusions in diamond gemstones. The researchers finally found what they were looking for in diamonds that are symmetrical across a central boundary. As these diamonds grew, a microscopic inclusion would sometimes become trapped along the boundary. Using a beam of electrons, the researchers identified 32 inclusions in eight of 30 diamonds they examined. Twenty of those inclusions were the same carbonate-bearing fluids found in the fibrous diamonds.
The finding suggests that while fibrous diamonds and gem-quality diamonds differ in age and price, they share common origins. “We’re coming full circle on the story,” Jablon says. “We can quiet a debate that has been raging in the field for a long time. Going forward, we can generally assume most diamonds crystalize the same way.”
A similar mechanism for creating older, gem-quality diamonds and younger, fibrous diamonds suggests that Earth has maintained diamond-forming conditions for billions of years, Stachel says. Carbonates are carried into Earth’s depths when tectonic plates subduct and sink into the planet’s interior. If ancient diamonds form from carbonates, plate tectonics could have already been churning the planet’s exterior 3.5 billion years ago, he says.